Unit 14: Muscle Physiology
Unit Outline
Part 1: The Neuromuscular Junction
Part 2: Skeletal muscle contraction and relaxation
- The Sliding Filament Model of contraction
- ATP and muscle contraction
- Sources of ATP: Creatine phosphate metabolism, the anaerobic pathway, and aerobic cellular respiration
- Classifying skeletal muscle
- Relaxation of a skeletal muscle
- Muscle tone
- Exercise and muscle performance
- Muscle atrophy
Practice Questions
Learning Outcomes
At the end of this unit, you should be able to:
I. Describe the anatomy of a neuromuscular junction.
II. Explain the process of muscle contraction and relaxation.
III. Contrast different types of skeletal muscle fibres.
IV. Explain how the nervous system controls muscle tension.
V. Describe the microscopic anatomy (histology) of cardiac muscle.
VI. Describe the mechanism of contraction in cardiac muscle.
VII. Describe the microscopic anatomy (histology) of smooth muscle.
VIII. Explain the mechanism of contraction and relaxation in smooth muscle.
Part 1: The Neuromuscular Junction
Two requirements must be met in order for skeletal muscular contraction to occur. First, there must be in place a neuromuscular junction.
The Neuromuscular Junction
The neuromuscular junction is the site where a motor neuron’s terminal meets a muscle fibre (the equivalent of a single muscle cell), and where that muscle fibre first responds to signaling by the motor neuron. Every skeletal muscle fibre must be activated, or stimulated, by a nerve ending at the neuromuscular junction so that a change in membrane potential occurs.
Excitation signals from the neuron are the only way to functionally activate the fibre to contract; they generate an electrical current, called an action potential, in the sarcolemma (plasma membrane) of the muscle fibre.
The action potential thus generated is linked to contraction, which is why the second requirement for skeletal muscular contraction is referred to as excitation-contraction coupling.
Excitation-Contraction Coupling
All living cells have membrane potentials, or electrical gradients across their membranes. The inside of the membrane is usually around -70 mV, relative to the outside. This is referred to as a cell’s resting membrane potential. Neurons and muscle cells can use their membrane potentials to generate electrical signals. They do this by controlling the movement of charged particles, called ions, across their membranes to create electrical currents. This is achieved by opening and closing specialized proteins in the membrane called ion channels. Although the currents generated by ions moving through these channel proteins are very small, they form the basis of both neural signaling and muscle contraction.
Both neurons and skeletal muscle cells are electrically excitable, meaning that they are able to generate action potentials. An action potential is a special type of electrical signal that can travel along a cell membrane as a wave. This allows a signal to be transmitted quickly and faithfully over long distances.
Although the term excitation-contraction coupling confuses or scares some students, it comes down to this: for a skeletal muscle fibre to contract, its membrane must first be “excited”—in other words, it must be stimulated to fire an action potential. The muscle fibre action potential, which sweeps along the sarcolemma as a wave, is “coupled” to the actual contraction through the release of calcium ions from the sarcoplasmic reticulum(SR).
In skeletal muscle, this sequence begins with signals from the somatic motor division of the nervous system. In other words, the “excitation” step in skeletal muscles is always triggered by signaling from the nervous system (Figure 1).
Signaling begins when a neuronal action potential travels along the axon of a motor neuron, and then along the individual branches of the axon, terminating at individual neuromuscular junctions. Membrane potential changes cause calcium channels in the membrane of the neuron to open, allowing calcium ions to diffuse into the neuron’s cytosol. This influx of Ca2+ causes vesicles in the neuron to fuse with its plasma membrane, releasing their contents – the neurotransmitter acetylcholine (ACh) – into the space between the neuron and the muscle fibre, called the synaptic cleft. It is the exocytotic release of acetylcholine from these vesicles that is ultimately calcium-ion dependent.
Thus, the associated axon terminal at each neuromuscular junction releases acetylcholine (ACh). The acetylcholine molecules diffuse across a minute space called the synaptic cleft and bind to acetylcholine receptors located within the motor end-plate of the sarcolemma on the other side of the synapse. Once acetylcholine binds, a channel in the acetylcholine receptor (called a ligand-gated ion channel) opens and positively charged ions can pass through into the muscle fibre, causing it to depolarize, meaning that the membrane potential of the muscle fibre becomes less negative (closer to zero, and this continues so that there is a temporary reversal of charge with the inside of the membrane briefly positive relative to the outside).
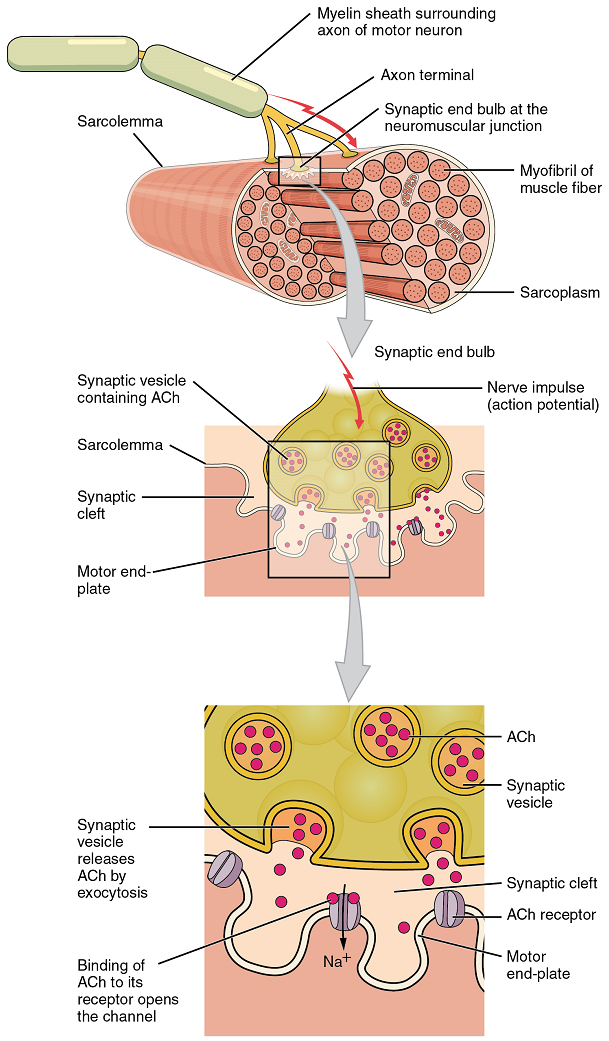
As the membrane depolarizes, another set of ion channels called voltage-gated sodium channels are triggered to open. Sodium ions enter the muscle fibre, and an action potential rapidly spreads (or “fires”) along the entire membrane to initiate excitation-contraction coupling.
Things happen very quickly in the world of excitable membranes (just think about how quickly you can snap your fingers as soon as you decide to do it). Immediately following depolarization of the membrane, it repolarizes, re-establishing the negative membrane potential. Meanwhile, the acetylcholine in the synaptic cleft is degraded by the enzyme acetylcholinesterase (AChE) so that the acetylcholine can no longer bind to an acetylcholine receptor, thereby avoiding unwanted extended muscle excitation and contraction.
Propagation of an action potential along the sarcolemma is still part of the excitation portion of excitation-contraction coupling; it is this excitation that triggers the release of calcium ions from its storage in the cell’s sarcoplasmic reticulum. For the action potential to reach the membrane of the SR, there are periodic invaginations that run deep within the sarcolemma, called transverse tubules (T-tubules). A T-tubule along with SR membranes on either side of it is referred to as a triad (Figure 2). Triads surround and enclose the cylindrical structure known as a myofibril, which contains actin and myosin.
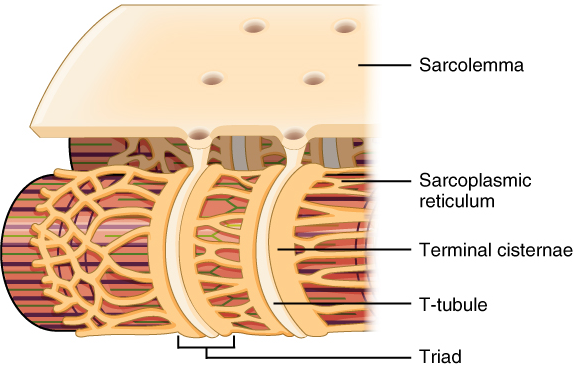
T-tubules thus carry the action potential into the interior of the cell. The action potential triggers the opening of calcium channels in the membrane of adjacent SRs, causing calcium ions to diffuse out of the SR and into the sarcoplasm. It is the arrival of Ca2+ in the sarcoplasm that initiates contraction of the muscle fibre by its contractile units, or sarcomeres.
Part 2: Skeletal Muscle Contraction and Relaxation
Once again, the sequence of events that result in the contraction of an individual muscle fibre begins with an electrical signal – an action potential – travelling down a motor neuron innervating the muscle fibre. Calcium’s role in initiating the release of acetylcholine from the motor neuron’s axon terminal has already been described.
When acetylcholine reaches the muscle fibre’s sarcolemma, it binds to closed acetylcholine -gated ion channels there that now open as a result. In the area where these ion channels open, the sarcolemma of the muscle fibre will depolarize as positively charged sodium ions (Na+) enter, triggering an action potential that spreads to the rest of the sarcolemma. The whole sarcolemma will depolarize, including the T-tubules.
Embedded in the walls of the T-tubules of skeletal muscle fibres are voltage-sensitive proteins that are connected to calcium channels in the membrane of the adjacent sarcoplasmic reticulum (SR). When the action potential travels along each T-tubule, the voltage-sensitive proteins there change shape, pulling on the calcium channels of the SR and opening them. This allows Ca2+ ions to be released from their storage in the SR (where their concentration is higher), out to the cytosol (sarcoplasm) of the muscle fibre. The Ca2+ ions then initiate contraction, which is sustained by ATP (Figure 3).
Troponin and tropomyosin are the two major proteins that regulate skeletal muscle contraction via Ca2+ ions binding. Tropomyosin is a long, rod-like molecule which shields (blocks) the myosin-binding sites on actin. Troponin, which has a binding site for Ca2+, is a globular protein whose role is to keep the longer, tropomyosin in its place.
Upon binding of Ca2+ to troponin, troponin changes its conformation (overall three-dimensional shape) and loses its hold on tropomyosin, thereby exposing the myosin-binding sites on actin.
Therefore, as long as calcium ions remain in the sarcoplasm to bind to troponin, which in turn keeps the myosin-binding sites on actin “unshielded,” and as long as ATP is available to drive the cross-bridge cycling and the pulling of actin strands by myosin, the muscle fibre will continue to shorten to an anatomical limit.
Muscle contraction usually stops when signaling from the motor neuron ends, which repolarizes the sarcolemma and T-tubules, and closes the voltage-gated calcium channels in the SR. Calcium ions are then pumped back into the SR, which causes the tropomyosin to reshield (or re-cover) the binding sites on the actin strands. A muscle also can stop contracting when it runs out of ATP and becomes fatigued (Figure 4).
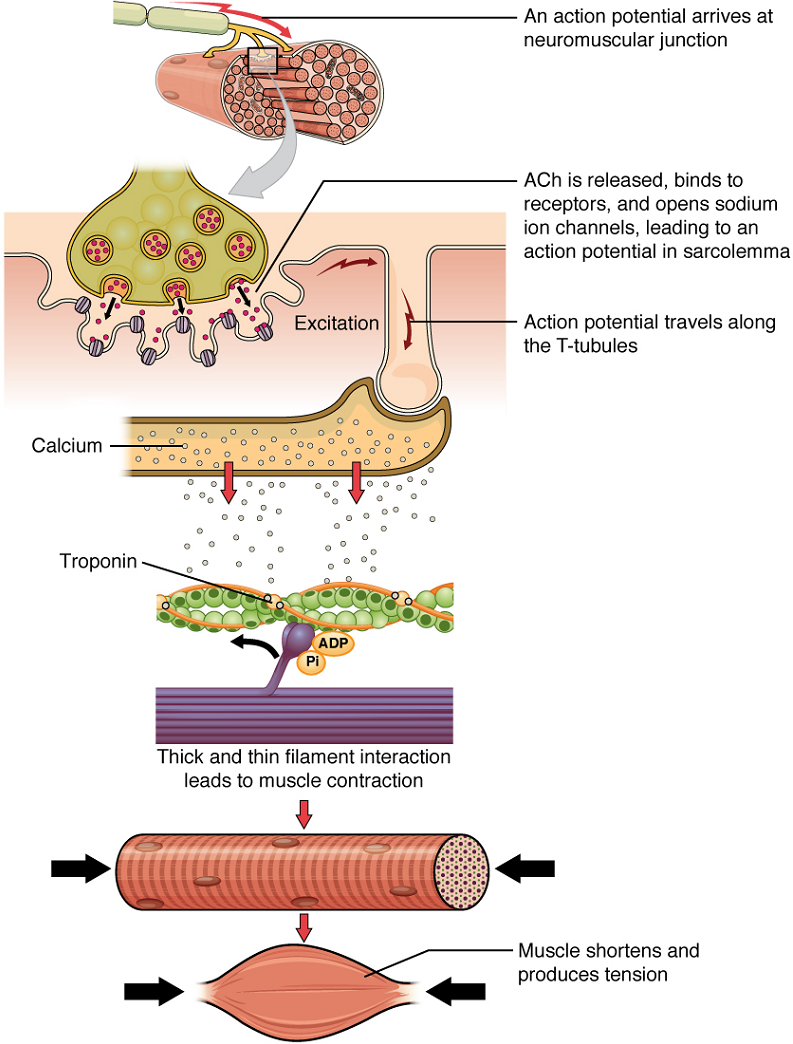
The molecular events of muscle fibre shortening occur within the fibre’s sarcomeres (Figure 5). The contraction of a striated muscle fibre occurs as the sarcomeres, linearly arranged within myofibrils, shorten as myosin heads pull on the actin filaments.
The region where thick and thin filaments overlap has a dense appearance, as there is little space between the filaments. This zone, where thin and thick filaments overlap, is very important to muscle contraction, as it is the site where filament movement starts. Thin filaments, anchored at their ends by the Z-discs, do not extend completely into the central region that only contains thick filaments, which are themselves anchored at their bases at a spot called the M-line. A myofibril is composed of many sarcomeres running along its length; thus, myofibrils and muscle cells shorten (contract) as the sarcomeres contract.
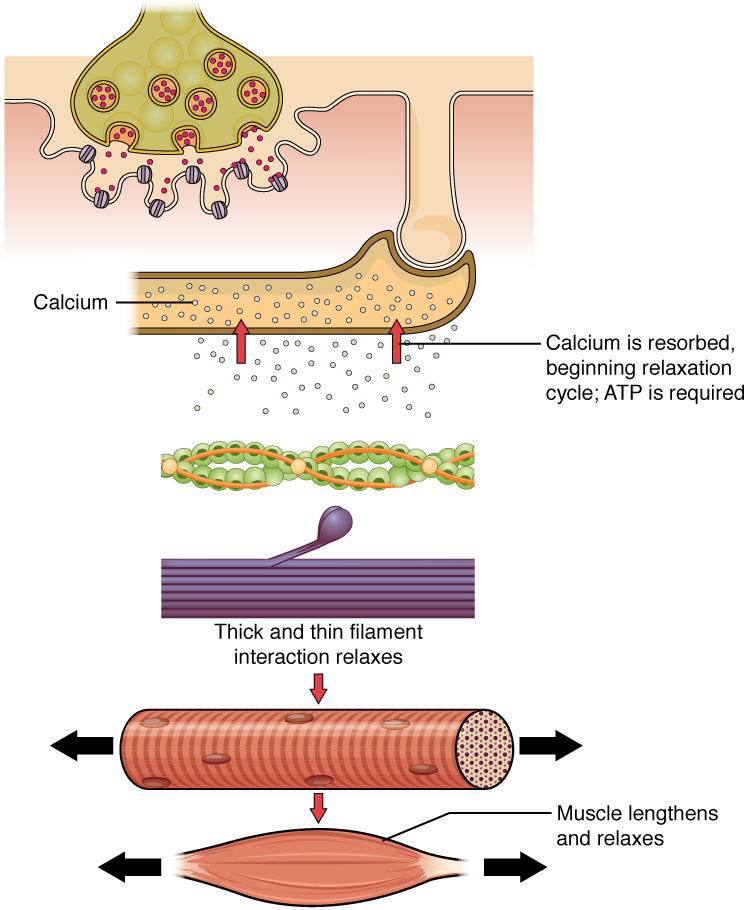
The Sliding Filament Model of Contraction
When signaled by a motor neuron, a skeletal muscle fibre contracts as the thin filaments are pulled and then slide past the thick filaments within the fibre’s sarcomeres. This process is known as the sliding filament model of muscle contraction (Figure 5). The sliding can only occur when myosin-binding sites on the actin filaments are exposed by a series of steps that begins with Ca2+ entry into the sarcoplasm.
Recall that to initiate muscle contraction, tropomyosin has to expose the myosin-binding site on an actin filament to allow cross-bridge formation between the actin and myosin microfilaments. The first step in the process of contraction is for Ca2+ to bind to troponin so that tropomyosin can slide away from the binding sites on the actin strands. This allows the myosin heads to bind to these exposed binding sites and form cross-bridges. The thin filaments are then pulled by the myosin heads to slide past the thick filaments toward the center of the sarcomere. But each head can only pull a very short distance before it has reached its limit and must be “re-cocked” before it can pull again, a step that requires ATP.
ATP and Muscle Contraction
For thin filaments to continue to slide past thick filaments during muscle contraction, myosin heads must pull the actin at the binding sites, detach, re-cock, attach to more binding sites, pull, detach, re-cock, etc. This repeated movement is known as the cross-bridge cycle.
Each cross-bridge cycle requires energy, which is provided for by ATP.
Cross-bridge formation occurs when the myosin head attaches to the actin while adenosine diphosphate (ADP) and inorganic phosphate (Pi) are still bound to myosin (Figure 6a,b). Pi is then released, causing myosin to form a stronger attachment to actin, after which the myosin head moves toward the M-line, pulling the actin along with it, and releasing the ADP. As actin is pulled, the filaments move approximately 10 nm toward the M-line. This movement is called the power stroke, as movement of the thin filament occurs at this step (Figure 6c). In the absence of ATP, the myosin head will not detach from actin.
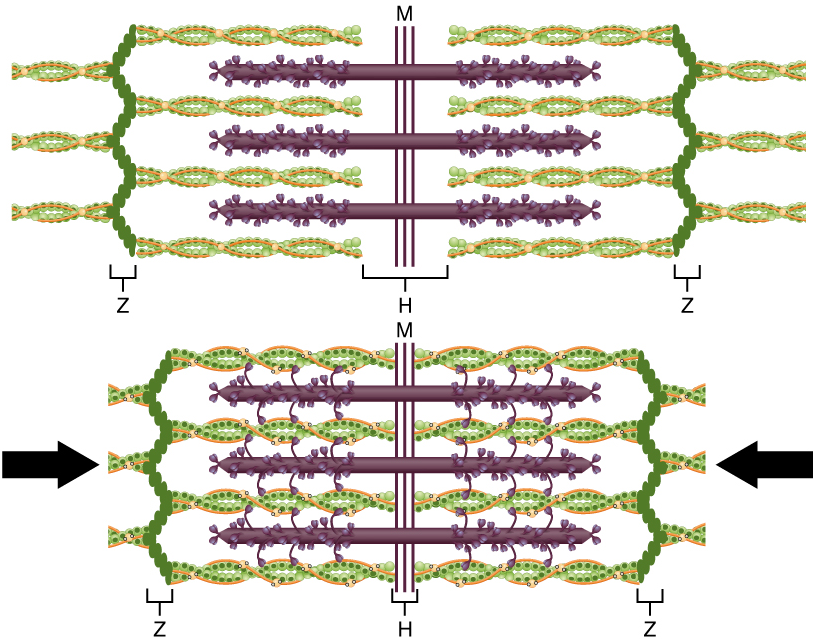
One part of the myosin head attaches to the binding site on the actin, but the head has another binding site for ATP. ATP binding causes the myosin head to detach from the actin (Figure 6d).
After this occurs, ATP is converted to ADP and Pi by the intrinsic ATPase activity of myosin. The energy released during ATP hydrolysis changes the angle of the myosin head into a cocked position (Figure 6e). When the myosin head is cocked, it is said to be in a high-energy configuration and is capable of further movement as long as ATP is available.
Note that each thick filament of roughly 300 myosin molecules has multiple myosin heads, and many cross-bridges form and break continuously during muscle contraction. Multiply this by all of the sarcomeres in one myofibril, all the myofibrils in one muscle fibre, and all of the muscle fibres in one skeletal muscle, and you can understand why so much energy (ATP) is needed to keep skeletal muscles working. In fact, it is the loss of ATP that results in the rigor mortis observed soon after someone dies. With no further ATP production possible, there is no ATP available for myosin heads to detach from the actin-binding sites, so the cross-bridges stay in place, causing the rigidity in the skeletal muscles.
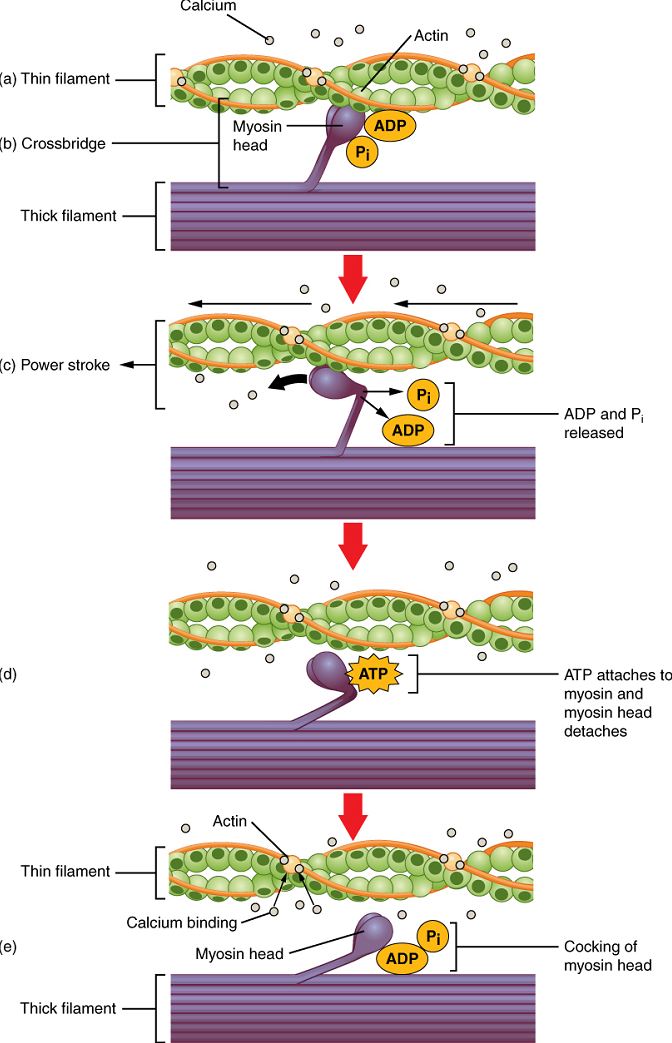
Relaxation of a Skeletal Muscle
Relaxing skeletal muscle fibres, and ultimately, the skeletal muscle, begins with the motor neuron, which stops releasing its chemical signal, acetylcholine, into the synapse at the neuromuscular junction. The muscle fibre will repolarize, which closes the gates in the SR where Ca2+ was being released. ATP-driven pumps will move Ca2+ out of the sarcoplasm back into the SR. Ca2+ no longer binds to troponin, resulting in the “reshielding” of the myosin-binding sites on the thin filaments by tropomyosin, which is now once again held in its place by troponin. Without the ability to form cross-bridges between the thin and thick filaments, the muscle fibre loses its tension and relaxes.
Sources of ATP
ATP supplies the energy for muscle contraction to take place. In addition to its direct role in the cross-bridge cycle, ATP also provides the energy for the active-transport utilizing Ca2+ pumps housed in the SR membranes. Muscle contraction does not occur without sufficient amounts of ATP. The amount of ATP stored in muscle is very low, only sufficient to power a few seconds worth of contractions.
Therefore, as it is broken down, ATP must be regenerated and replaced quickly to allow for sustained contraction: ATP can be regenerated through three mechanisms: creatine phosphate metabolism, anaerobic pathway (glycolysis and lactic acid formation) and aerobic cellular respiration.
(a) Creatine phosphate is a molecule that can store energy in its phosphate bonds. In a resting muscle, excess ATP transfers its energy to creatine, producing ADP and creatine phosphate. This acts as an energy reserve that can be used to quickly create more ATP. When the muscle starts to contract and needs energy, creatine phosphate transfers its phosphate back to ADP to form ATP and creatine. This reaction is catalyzed by the enzyme creatine kinase and occurs very quickly; thus, creatine phosphate-derived ATP powers the first few seconds of muscle contraction. However, creatine phosphate can only provide approximately 15 seconds worth of energy, at which point another energy source has to be used (Figure 7).
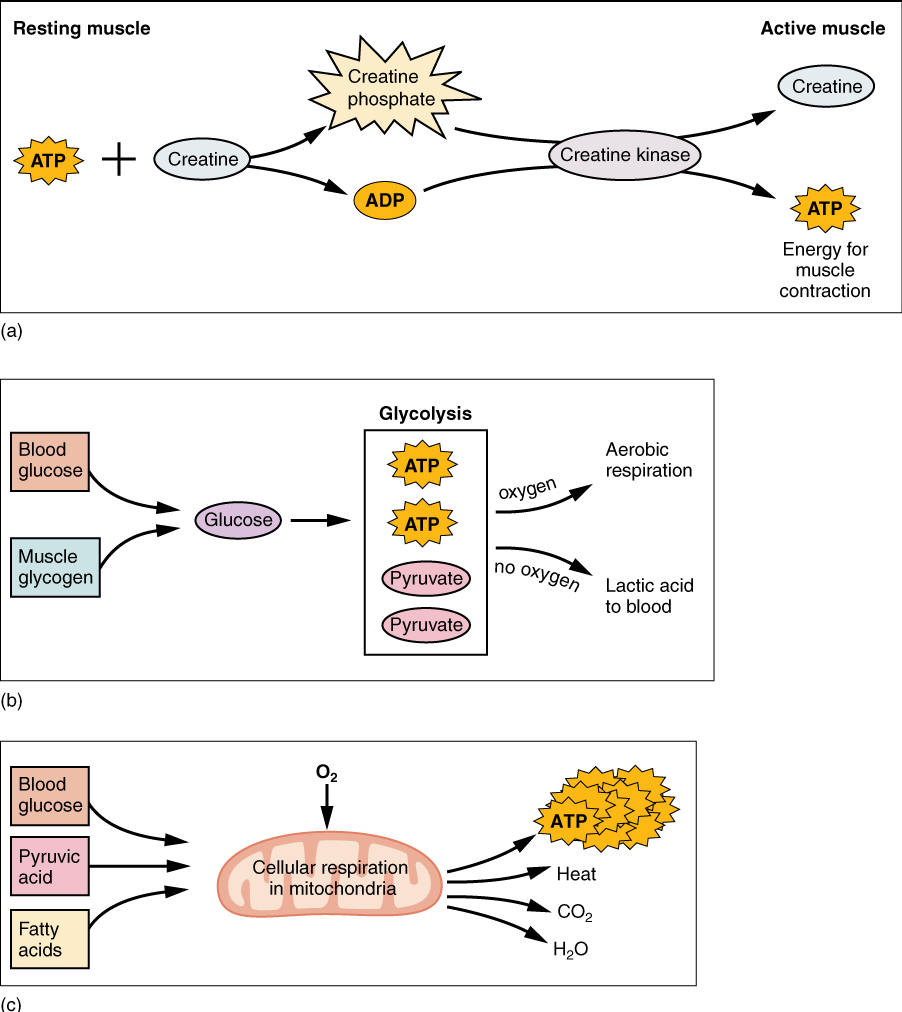
(b) The Anaerobic Pathway: As the ATP produced by creatine phosphate is depleted, muscles turn to glycolysis as an ATP source. Glycolysis is an anaerobic (non-oxygen-dependent) process that breaks down glucose (sugar) to produce ATP. Because glycolysis cannot generate ATP as quickly as creatine phosphate, the switch to glycolysis results in a slower rate of ATP availability to the muscle. The sugar used in glycolysis can be provided by blood glucose or by metabolizing glycogen that is stored in the muscle. The breakdown of one glucose molecule produces two ATP and two molecules of pyruvic acid, which can be used either in aerobic respiration if sufficient oxygen is available, or when oxygen levels are low, converted to lactic acid (Figure 7b).
The lactic acid so produced may contribute to muscle fatigue. This conversion allows the recycling of the coenzyme NAD+ from NADH, which is needed for glycolysis to continue. This occurs during strenuous exercise when high amounts of energy are needed but oxygen cannot be sufficiently delivered to muscle. Glycolysis itself cannot be sustained for very long (approximately one minute of muscle activity), but it is useful in facilitating short bursts of high-intensity output. This is because glycolysis does not utilize glucose very efficiently, producing a net gain of two ATPs per molecule of glucose, and the end product of lactic acid.
(c) Aerobic cellular respiration is the breakdown of glucose or other nutrients in the presence of oxygen to produce carbon dioxide, water, and ATP. Approximately 95 percent of the ATP required for resting or moderately active muscles is provided by aerobic respiration, which takes place in mitochondria. The inputs for aerobic respiration include glucose circulating in the bloodstream, pyruvic acid, and fatty acids. Aerobic respiration is much more efficient than anaerobic glycolysis, producing approximately 32 to 34 ATPs per molecule of glucose versus two (net) from glycolysis. However, aerobic respiration cannot be sustained without a steady supply of O2 to the skeletal muscle (Figure 7c). To compensate, muscles store small amount of excess oxygen in a protein called myoglobin, allowing for more efficient muscle contractions and less fatigue. Aerobic training also increases the efficiency of the circulatory system so that O2 can be supplied to the muscles for longer periods of time.
Classifying Skeletal Muscle
Two criteria to consider when classifying the types of muscle fibres are how fast some fibres contract relative to others, and how fibres produce ATP. Using these criteria, there are three main types of skeletal muscle fibres. Slow oxidative (SO) fibres contract relatively slowly and use aerobic respiration (oxygen and glucose) to produce ATP. Fast oxidative (FO) fibres have fast contractions and primarily use aerobic respiration, but because they may switch to anaerobic respiration (glycolysis), can fatigue more quickly than SO fibres. Lastly, fast glycolytic (FG) fibres have fast contractions and primarily use anaerobic glycolysis. The FG fibres fatigue more quickly than the others. Most skeletal muscles in a human contain(s) all three types, although in varying proportions.
The speed of contraction is dependent on how quickly myosin’s ATPase hydrolyzes ATP to produce cross-bridge action. Fast fibres hydrolyze ATP approximately twice as quickly as slow fibres, resulting in much quicker cross-bridge cycling (which pulls the thin filaments toward the center of the sarcomeres at a faster rate). The primary metabolic pathway used by a muscle fibre determines whether the fibre is classified as oxidative or glycolytic. If a fibre primarily produces ATP through aerobic pathways it is oxidative. More ATP can be produced during each metabolic cycle, making the fibre more resistant to fatigue. Glycolytic fibres primarily create ATP through anaerobic glycolysis, which produces less ATP per cycle. As a result, glycolytic fibres fatigue at a quicker rate.
The oxidative fibres contain many more mitochondria than the glycolytic fibres, because aerobic metabolism, which uses oxygen (O2) in the metabolic pathway, occurs in the mitochondria. The SO fibres possess a large number of mitochondria and are capable of contracting for longer periods because of the large amount of ATP they can produce, but they have a relatively small diameter and do not produce a large amount of tension. SO fibres are extensively supplied with blood capillaries to supply O2 from the red blood cells in the bloodstream. The SO fibres also possess myoglobin, an O2-carrying molecule similar to O2-carrying hemoglobin in the red blood cells. The myoglobin stores some of the needed O2 within the fibres themselves (and gives SO fibres their red color). All of these features allow SO fibres to produce large quantities of ATP, which can sustain muscle activity without fatiguing for long periods of time.
The fact that SO fibres can function for long periods without fatiguing makes them useful in maintaining posture, producing isometric contractions, stabilizing bones and joints, and making small movements that happen often but do not require large amounts of energy. They do not produce high tension, and thus they are not used for powerful, fast movements that require high amounts of energy and rapid cross-bridge cycling.
FO fibres are sometimes called intermediate fibres because they possess characteristics that are intermediate between fast fibres and slow fibres. They produce ATP relatively quickly, more quickly than SO fibres, and thus can produce relatively high amounts of tension. They are oxidative because they produce ATP aerobically, possess high amounts of mitochondria, and do not fatigue quickly. However, FO fibres do not possess significant myoglobin, giving them a lighter color than the red SO fibres. FO fibres are used primarily for movements, such as walking, that require more energy than postural control but less energy than an explosive movement, such as sprinting. FO fibres are useful for this type of movement because they produce more tension than SO fibres but they are more fatigue-resistant than FG fibres.
FG fibres primarily use anaerobic glycolysis as their ATP source. They have a large diameter and possess high amounts of glycogen, which is used in glycolysis to generate ATP quickly to produce high levels of tension. Because they do not primarily use aerobic metabolism, they do not possess substantial numbers of mitochondria or significant amounts of myoglobin and therefore have a white color. FG fibres are used to produce rapid, forceful contractions to make quick, powerful movements. These fibres fatigue quickly, permitting them to only be used for short periods. Most muscles possess a mixture of each fibre type. The predominant fibre type in a muscle is determined by the primary function of the muscle.
Nervous System Control of Muscle Tension
To move an object, referred to as load, the sarcomeres in the muscle fibres of the skeletal muscle must shorten. The force generated by the contraction of the muscle (or shortening of the sarcomeres) is called muscle tension. However, muscle tension also is generated when the muscle is contracting against a load that does not move, resulting in two main types of skeletal muscle contractions: isotonic contractions and isometric contractions.
In isotonic contractions, where the tension in the muscle stays constant, a load is moved as the length of the muscle changes (shortens). There are two types of isotonic contractions: concentric and eccentric. A concentric contraction involves the muscle shortening to move a load. An example of this is the biceps brachii muscle contracting when a hand weight is brought upward with increasing muscle tension. As the biceps brachii contract, the angle of the elbow joint decreases as the forearm is brought toward the body. Here, the biceps brachii contracts as sarcomeres in its muscle fibres are shortening and cross-bridges form; the myosin heads pull the actin. An eccentric contraction occurs as the muscle tension diminishes and the muscle lengthens. In this case, the hand weight is lowered in a slow and controlled manner as the amount of cross-bridges being activated by nervous system stimulation decreases. In this case, as tension is released from the biceps brachii, the angle of the elbow joint increases. Eccentric contractions are also used for movement and balance of the body.
An isometric contraction occurs as the muscle produces tension without changing the angle of a skeletal joint. Isometric contractions involve sarcomere shortening and increasing muscle tension, but do not move a load, as the force produced cannot overcome the resistance provided by the load. For example, if one attempts to lift a hand weight that is too heavy, there will be sarcomere activation and shortening to a point, and ever-increasing muscle tension, but no change in the angle of the elbow joint. In everyday living, isometric contractions are active in maintaining posture and maintaining bone and joint stability. However, holding your head in an upright position occurs not because the muscles cannot move the head, but because the goal is to remain stationary and not produce movement. Most actions of the body are the result of a combination of isotonic and isometric contractions working together to produce a wide range of outcomes (Figure 8).
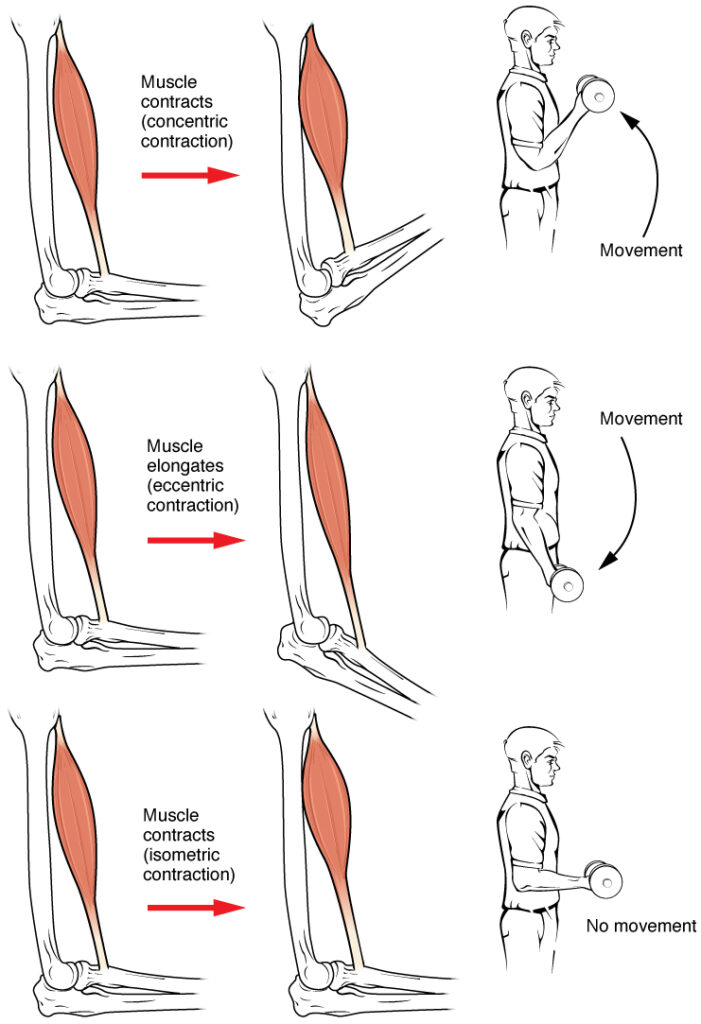
All of these muscle activities are under the exquisite control of the nervous system. Neural control regulates concentric, eccentric and isometric contractions, muscle fibre recruitment, and muscle tone. A crucial aspect of nervous system control of skeletal muscles is the role of motor units.
Motor Units
As you have learned, every skeletal muscle fibre must be innervated by the axon terminal of a motor neuron in order to contract. Each muscle fibre is innervated by only one motor neuron. The actual group of muscle fibres in a muscle innervated by a single motor neuron is called a motor unit. The size of a motor unit is variable depending on the nature of the muscle.
A small motor unit is an arrangement where a single motor neuron supplies a small number of muscle fibres in a muscle. Small motor units permit very fine motor control of the muscle. The best example in humans is the small motor units of the extraocular eye muscles that move the eyeballs. There are thousands of muscle fibres in each muscle, but every six or so fibres are supplied by a single motor neuron, as the axons branch to form synaptic connections at their individual NMJs. This allows for exquisite control of eye movements so that both eyes can quickly focus on the same object. Small motor units are also involved in the many fine movements of the fingers and thumb of the hand for grasping, texting, etc.
A large motor unit is an arrangement where a single motor neuron supplies a large number of muscle fibres in a muscle. Large motor units are concerned with simple, or “gross,” movements, such as powerfully extending the knee joint. The best example is the large motor units of the thigh muscles or back muscles, where a single motor neuron will supply thousands of muscle fibres in a muscle, as its axon splits into thousands of branches.
There is a wide range of motor units within many skeletal muscles, which gives the nervous system a wide range of control over the muscle. The small motor units in the muscle will have smaller, lower-threshold motor neurons that are more excitable, firing first to their skeletal muscle fibres, which also tend to be the smallest. Activation of these smaller motor units, results in a relatively small degree of contractile strength (tension) generated in the muscle. As more strength is needed, larger motor units, with bigger, higher-threshold motor neurons are enlisted to activate larger muscle fibres. This increasing activation of motor units produces an increase in muscle contraction known as recruitment. As more motor units are recruited, the muscle contraction grows progressively stronger. In some muscles, the largest motor units may generate a contractile force of 50 times more than the smallest motor units in the muscle. This allows a feather to be picked up using the biceps brachii arm muscle with minimal force, and a heavy weight to be lifted by the same muscle by recruiting the largest motor units.
When necessary, the maximal number of motor units in a muscle can be recruited simultaneously, producing the maximum force of contraction for that muscle, but this cannot last for very long because of the energy requirements to sustain the contraction. To prevent complete muscle fatigue, motor units are generally not all simultaneously active, but instead some motor units rest while others are active, which allows for longer muscle contractions. The nervous system uses recruitment as a mechanism to efficiently utilize a skeletal muscle.
The Length-Tension Range of a Sarcomere
When a skeletal muscle fibre contracts, myosin heads attach to actin to form cross-bridges followed by the thin filaments sliding over the thick filaments as the heads pull the actin, and this results in sarcomere shortening, creating the tension of the muscle contraction. The cross-bridges can only form where thin and thick filaments already overlap, so that the length of the sarcomere has a direct influence on the force generated when the sarcomere shortens. This is called the length-tension relationship.
The ideal length of a sarcomere to produce maximal tension occurs at 80 percent to 120 percent of its resting length, with 100 percent being the state where the medial edges of the thin filaments are just at the most-medial myosin heads of the thick filaments (Figure 9). This length maximizes the overlap of actin-binding sites and myosin heads. If a sarcomere is stretched past this ideal length (beyond 120 percent), thick and thin filaments do not overlap sufficiently, which results in less tension produced. If a sarcomere is shortened beyond 80 percent, the zone of overlap is reduced with the thin filaments jutting beyond the last of the myosin heads and shrinks the H zone, which is normally composed of myosin tails. Eventually, there is nowhere else for the thin filaments to go and the amount of tension is diminished. If the muscle is stretched to the point where thick and thin filaments do not overlap at all, no cross-bridges can be formed, and no tension is produced in that sarcomere. This amount of stretching does not usually occur, as accessory proteins and connective tissue oppose extreme stretching.
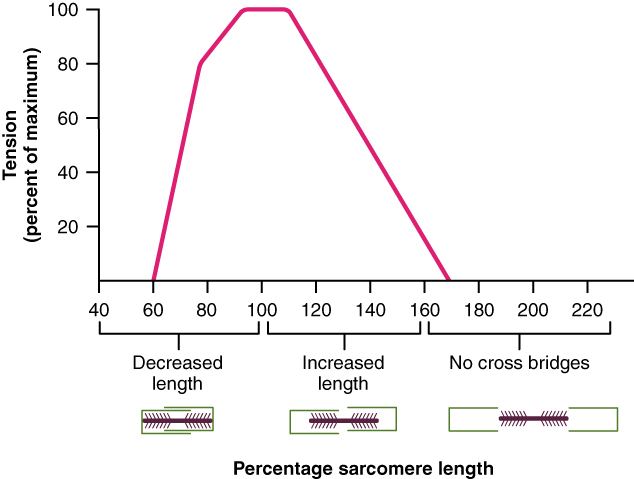
The Frequency of Motor Neuron Stimulation
A single action potential from a motor neuron will produce a single contraction in the muscle fibres of its motor unit. This isolated contraction is called a twitch. A twitch can last for a few milliseconds or 100 milliseconds, depending on the muscle type. The tension produced by a single twitch can be measured by a myogram, an instrument that measures the amount of tension produced over time (Figure 10). Each twitch undergoes three phases. The first phase is the latent period, during which the action potential is being propagated along the sarcolemma and Ca++ ions are released from the SR. This is the phase during which excitation and contraction are being coupled but contraction has yet to occur. The contraction phase occurs next. The Ca++ ions in the sarcoplasm have bound to troponin, tropomyosin has shifted away from actin-binding sites, cross-bridges have formed, and sarcomeres are actively shortening to the point of peak tension. The last phase is the relaxation phase, when tension decreases as contraction stops. Ca++ ions are pumped out of the sarcoplasm into the SR, and cross-bridge cycling stops, returning the muscle fibres to their resting state.
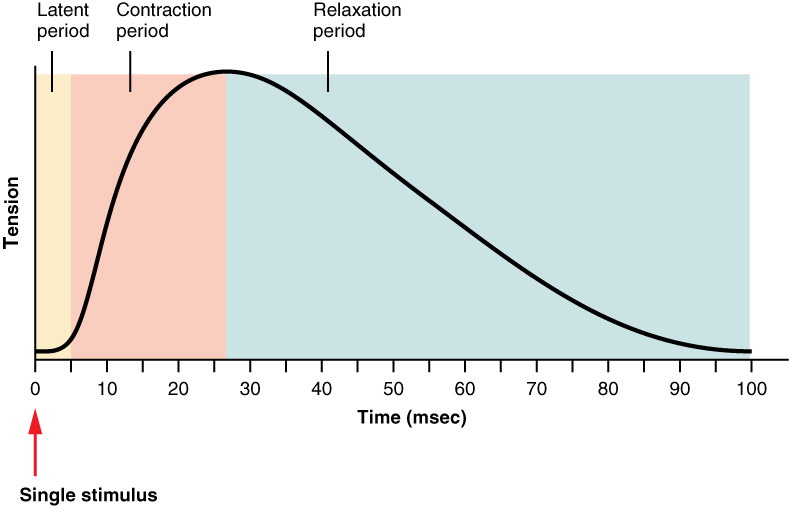
Although a person can experience a muscle “twitch,” a single twitch does not produce any significant muscle activity in a living body. A series of action potentials to the muscle fibres is necessary to produce a muscle contraction that can produce work. Normal muscle contraction is more sustained, and it can be modified by input from the nervous system to produce varying amounts of force; this is called a graded muscle response. The frequency of action potentials (nerve impulses) from a motor neuron and the number of motor neurons transmitting action potentials both affect the tension produced in skeletal muscle.
The rate at which a motor neuron fires action potentials affects the tension produced in the skeletal muscle. If the fibres are stimulated while a previous twitch is still occurring, the second twitch will be stronger. This response is called wave summation, because the excitation-contraction coupling effects of successive motor neuron signaling is summed, or added together (Figure 11a). At the molecular level, summation occurs because the second stimulus triggers the release of more Ca++ ions, which become available to activate additional sarcomeres while the muscle is still contracting from the first stimulus. Summation results in greater contraction of the motor unit.
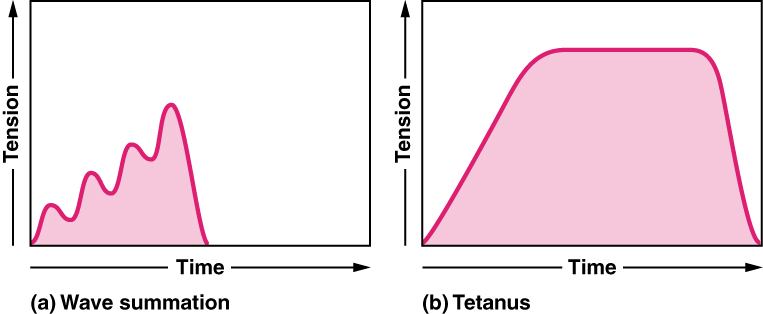
If the frequency of motor neuron signaling increases, summation and subsequent muscle tension in the motor unit continues to rise until it reaches a peak point. The tension at this point is about three to four times greater than the tension of a single twitch, a state referred to as incomplete tetanus. During incomplete tetanus, the muscle goes through quick cycles of contraction with a short relaxation phase for each. If the stimulus frequency is so high that the relaxation phase disappears completely, contractions become continuous in a process called complete tetanus (Figure 11b).
During tetanus, the concentration of Ca++ ions in the sarcoplasm allows virtually all of the sarcomeres to form cross-bridges and shorten, so that a contraction can continue uninterrupted (until the muscle fatigues and can no longer produce tension).
Muscle Tone
Skeletal muscles are rarely completely relaxed, or flaccid. Even if a muscle is not producing movement, it is contracted a small amount to maintain its contractile proteins and produce muscle tone. This continuous partial contraction of a muscle that causes the muscle to resist passive stretch while at rest is referred to as muscle tone. The tension produced by muscle tone allows muscles to continually stabilize joints and maintain posture.
Muscle tone is accomplished by a complex interaction between the nervous system and skeletal muscles that results in the activation of a few motor units at a time, most likely in a cyclical manner. In this manner, muscles never fatigue completely, as some motor units can recover while others are active.
Exercise and Muscle Performance
Physical training alters the appearance of skeletal muscles and can produce changes in muscle performance. Conversely, a lack of use can result in decreased performance and muscle appearance. Although muscle cells can change in size, new cells are not formed when muscles grow. Instead, structural proteins are added to muscle fibres in a process called hypertrophy, so cell diameter increases. The reverse, when structural proteins are lost and muscle mass decreases, is called atrophy. Age-related muscle atrophy is called sarcopenia. Cellular components of muscles can also undergo changes in response to changes in muscle use.
Muscle Atrophy
Although atrophy due to disuse can often be reversed with exercise, muscle atrophy can also be the result of any of a number of genetic diseases, called muscular dystrophy, that result in increasing weakness of muscles and loss of muscle tissue over time. Although there are medications that can slow muscle degeneration and reduce damage to dying muscle cells, the atrophy due to muscular dystrophy is irreversible. Muscle atrophy with age, referred to as sarcopenia, is also irreversible. This is a primary reason why even highly trained athletes succumb to declining performance with age. This decline is noticeable in athletes whose sports require strength and powerful movements, such as sprinting, whereas the effects of age are less noticeable in endurance athletes such as marathon runners or long-distance cyclists. As muscles age, muscle fibres die, and they are replaced by connective tissue and adipose tissue (Figure 8). Because those tissues cannot contract and generate force as muscle can, muscles lose the ability to produce powerful contractions. The decline in muscle mass causes a loss of strength, including the strength required for posture and mobility. This may be caused by a reduction in FG fibres that hydrolyze ATP quickly to produce short, powerful contractions. Muscles in older people sometimes possess greater numbers of SO fibres, which are responsible for longer contractions and do not produce powerful movements. There may also be a reduction in the size of motor units, resulting in fewer fibres being stimulated and less muscle tension being produced.
Sarcopenia can be delayed to some extent by exercise, as training adds structural proteins and causes cellular changes that can offset the effects of atrophy. Increased exercise can produce greater numbers of cellular mitochondria, increase capillary density, and increase the mass and strength of connective tissue.
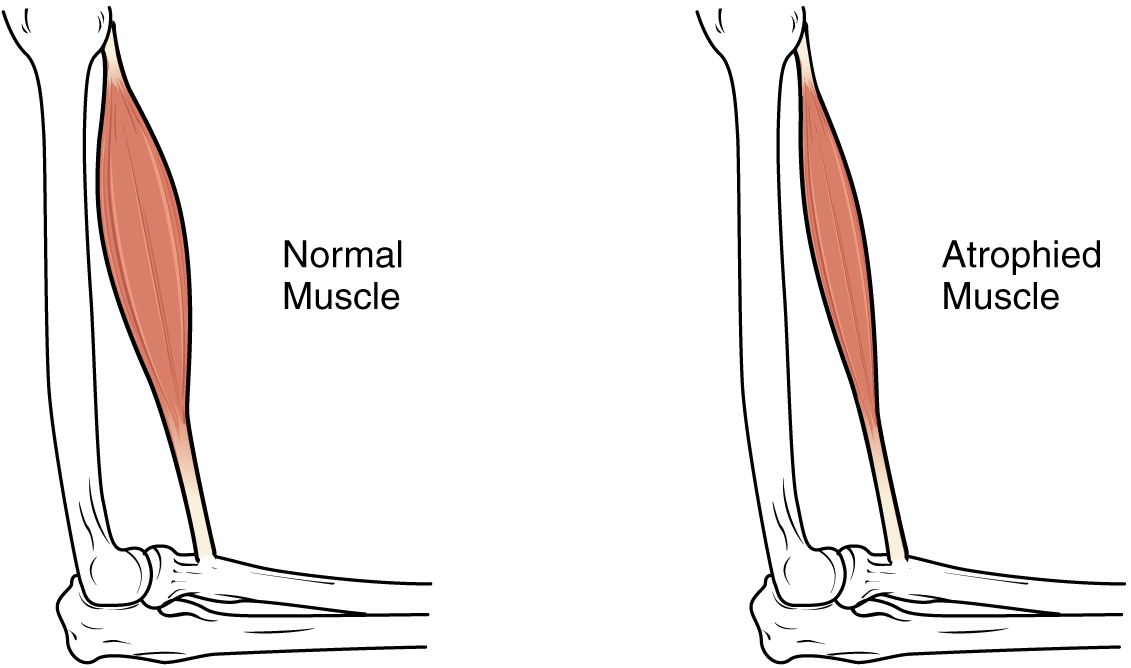
The effects of age-related atrophy are especially pronounced in people who are sedentary, as the loss of muscle cells is displayed as functional impairments such as trouble with locomotion, balance, and posture. This can lead to a decrease in quality of life and medical problems, such as joint problems because the muscles that stabilize bones and joints are weakened. Problems with locomotion and balance can also cause various injuries due to falls.
Part 3: Cardiac Muscle Tissue
Cardiac muscle tissue is only found in the heart. Highly coordinated contractions of cardiac muscle pump blood into the vessels of the circulatory system. Similar to skeletal muscle, cardiac muscle is striated and organized into sarcomeres, possessing the same banding organization as skeletal muscle (Figure 13). However, cardiac muscle fibres are shorter than skeletal muscle fibres and many contain only one nucleus, which is located in the central region of the cell, though a considerable number are also bi- or multinucleated. Cardiac muscle fibres, similarly to skeletal muscle fibres, also possess many mitochondria and myoglobin, as ATP is produced primarily through aerobic metabolism. Cardiac muscle fibre cells are also extensively branched and are connected to one another at their ends by intercalated discs. An intercalated disc allows the cardiac muscle cells to contract in a wave-like pattern so that the heart can work as a pump.
Gap Junctions and Desmosomes
Intercalated discs are part of the sarcolemma and contain two structures important in cardiac muscle contraction: gap junctions and desmosomes. A gap junction forms channels between adjacent cardiac muscle fibres that allow the depolarizing current produced by cations to flow from one cardiac muscle cell to the next. This joining is called electric coupling (as opposed to excitation-contraction coupling), and in cardiac muscle it allows the quick transmission of action potentials and the coordinated contraction of the entire heart. This network of electrically connected cardiac muscle cells creates a functional unit of contraction called a syncytium. The remainder of the intercalated disc is composed of desmosomes. A desmosome is a cell structure that anchors the ends of cardiac muscle fibres together so the cells do not pull apart during the stress of individual fibres contracting (Figure 14).
Electrical Coupling
Contractions of the heart (heartbeats) are controlled by specialized cardiac muscle cells called pacemaker cells that directly control heart rate. Although cardiac muscle cannot be consciously controlled, the pacemaker cells respond to signals from the autonomic nervous system to increase or decrease heart rate. The pacemaker cells can also respond to various hormones with the effect of modulating heart rate and thus also controlling blood pressure.
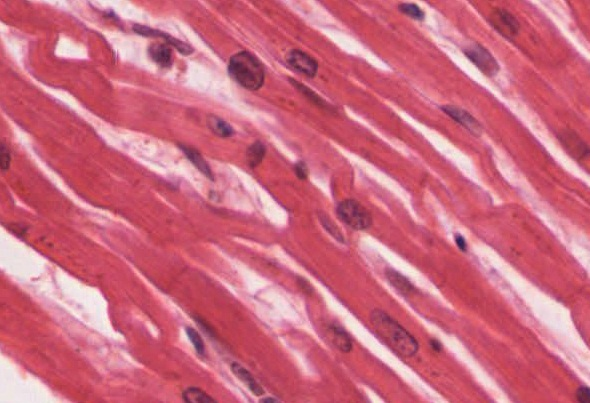
The Functional Syncytium
The functional syncytium (the wave of contraction that allows the heart to work as a unit) begins with the pacemaker cells. This group of cells is self-excitable and able to depolarize to threshold and fire action potentials on their own, a feature called autorhythmicity; they do this at set intervals which determine heart rate. Because they are connected with gap junctions to surrounding muscle fibres and the specialized fibres of the heart’s conduction system, the pacemaker cells are able to transfer the depolarization to the other cardiac muscle fibres in a manner that allows the heart to contract in a coordinated manner.
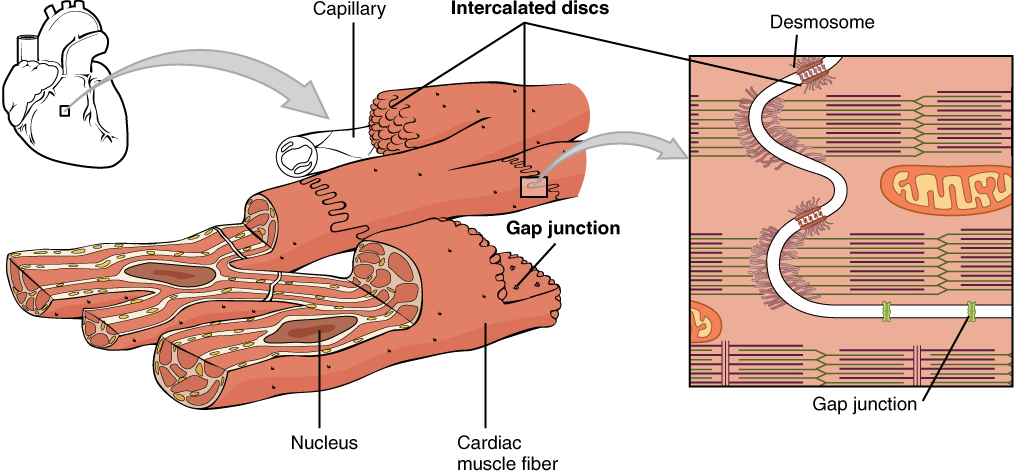
In cardiac cells, unlike skeletal muscles, extracellular Ca2+ is required to initiate release of calcium from the sarcoplasmic reticulum (SR). The SR in cardiac muscle fibres is simpler than that of skeletal muscle fibres, lacking terminal cisterns, and there is no direct physical link between proteins in the T-tubule and proteins in the SR membrane, so depolarization of the T-tubule membrane cannot directly cause Ca2+ release from the SR. Instead, cardiac muscle cells have voltage-gated calcium channels in the sarcolemma and along the T-tubules that open when the membrane is depolarized, allowing Ca2+ to enter the cardiac muscle fibre from the extracellular fluid. This calcium then causes the opening of calcium-gated calcium channels in the SR membrane that release additional Ca2+ into the sarcoplasm. This mechanism allows cardiac muscle to have a relatively long-lasting depolarization “plateau” in its fibres. This sustained depolarization (and Ca2+ entry) provides for a longer contraction than is produced by an action potential in skeletal muscle.
Part 4: Smooth Muscle Tissue
Smooth muscle (Figure 15), so named because the cells do not have striations, is present in the walls of hollow organs like the urinary bladder, uterus, stomach, intestines, and in the walls of passageways, such as the arteries and veins of the circulatory system, and the tracts of the respiratory, urinary, and reproductive systems. Smooth muscle is also present in the eyes, where it functions to change the size of the iris and alter the shape of the lens. It is also present in the skin where it causes hair to stand erect in response to cold temperature or fear.
Smooth muscle fibres are spindle-shaped (wide in the middle and tapered at both ends, somewhat like a football) and have a single nucleus; they range from about 30 to 200 μm (thousands of times shorter than skeletal muscle fibres). Although they do not have striations and sarcomeres, smooth muscle fibres do have thick and thin filaments composed of myosin and actin contractile proteins. These thin filaments are anchored by dense bodies. A dense body is analogous to the Z-discs of skeletal and cardiac muscle fibres and is tethered, or fastened, to the sarcolemma. Calcium ions are supplied by the sarcoplasmic reticulum (SR) in the fibres and by sequestration from the extracellular fluid through membrane indentations called caveolae.
Because smooth muscle cells do not contain troponin, cross-bridge formation is not regulated by the troponin-tropomyosin complex but instead by the regulatory protein calmodulin. In a smooth muscle fibre, external calcium ions passing through opened calcium channels in the sarcolemma, and additional Ca2+ released from SR, bind to calmodulin. The Ca2+-calmodulin complex then activates an enzyme called myosin (light chain) kinase, which, in turn, activates the myosin heads by phosphorylating them (converting ATP to ADP and Pi, with the Pi attaching to the head). The myosin heads can then attach to actin-binding sites and pull on the thin filaments. The thin filaments are anchored to the dense bodies, which also have cord-like intermediate filaments attached to them. In fact, intermediate filaments appear as a network throughout the sarcoplasm and are connected to each other through dense bodies. Thus, as the thin filaments slide past the thick filaments, they pull on the dense bodies, which in turn pull on the network of intermediate filaments throughout the sarcoplasm. This arrangement causes the entire muscle fibre to contract in a manner which sees its ends being pulled toward the center, causing the midsection to bulge inward, like a corkscrew (Figure 16).
Although smooth muscle contraction relies on the presence of calcium ions, smooth muscle fibres have a much smaller diameter than skeletal muscle cells. Smooth muscle fibres have a limited calcium-storing SR but have calcium channels in the sarcolemma (similar to cardiac muscle fibres) that open during the action potential along the sarcolemma. The influx of extracellular calcium ions, which diffuse into the sarcoplasm to reach the calmodulin, accounts for most of the Ca2+ that triggers contraction of a smooth muscle cell.
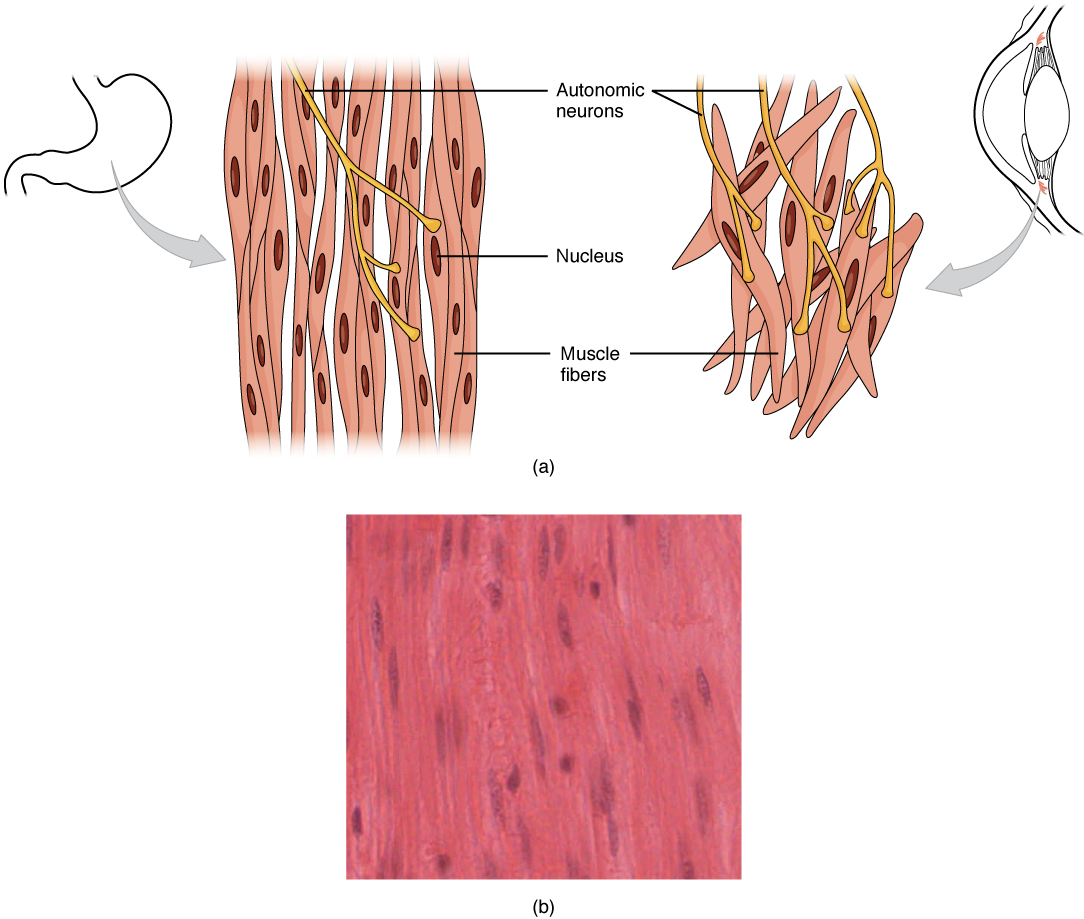
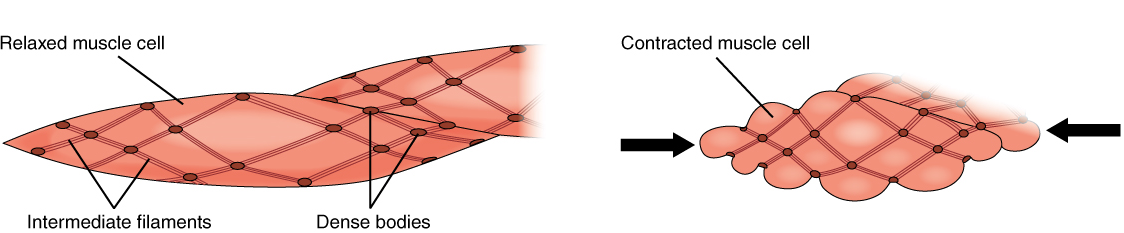
Muscle contraction continues until ATP-dependent calcium pumps actively transport calcium ions back into the SR and out of the cell. However, a low concentration of calcium remains in the sarcoplasm to maintain muscle tone. This remaining calcium keeps the muscle slightly contracted, which is important in certain tracts and around blood vessels.
Because most smooth muscles must function for long periods without rest, their power output is relatively low, but contractions can continue without using large amounts of energy. Some smooth muscle can also maintain contractions even as Ca2+ is removed and myosin kinase is inactivated/dephosphorylated. This can happen because a subset of cross-bridges between myosin heads and actin, called latch-bridges, keep the thick and thin filaments linked together for a prolonged period, and without the need for ATP. This allows for the maintaining of muscle “tone” in smooth muscle that lines arterioles and other visceral organs with very little energy expenditure.
Smooth muscle is not under voluntary control; thus, it is called involuntary muscle. The triggers for smooth muscle contraction include hormones, neural stimulation by the autonomic nervous system, and local factors (e.g. localized histamine release, pH levels etc).
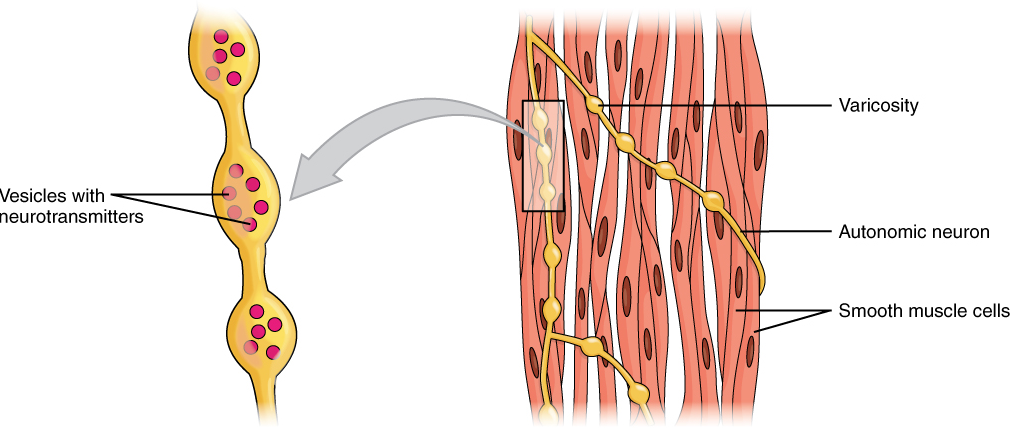
Different autonomic nerves release various neurotransmitters onto smooth muscle. For example, some nerves release acetylcholine that causes contraction of smooth muscle around some respiratory ducts and thus constriction of these airways. Other nerves release norepinephrine that causes relaxation of smooth muscle and thus widening of the airways. The same neurotransmitter can even cause opposite effects depending partly on the tissue where it acts and/or the variant of neurotransmitter receptor on target cells. Although norepinephrine causes relaxation of smooth muscle and thus widening of some airways, it also causes contraction of smooth muscle and thus constriction of most blood vessels. Autonomic neurons innervating smooth muscle release their neurotransmitters from swellings along their axons, called varicosities, that tend to result in less specific localization of the released neurotransmitter than at a neuromuscular junction (Figure 17).
Several hormones also affect the activity of smooth muscle, either by encouraging contraction or relaxation. For example, in the digestive system, cholecystokinin induces relaxation of the smooth muscle around the hepatopancreatic sphincter causing it to open. Conversely, gastrin stimulates contraction of smooth muscle in the stomach to enhance the churning activity of the stomach. Within the reproductive system, oxytocin stimulates uterine smooth muscle contraction to facilitate childbirth.
Smooth muscle arranged in layers around a hollow organ generally produces slow, steady contractions known as peristalsis that allow substances, such as food in the digestive tract, to move through the body. One layer of smooth muscle is parallel to the longitudinal axis of the lumen and the other layer is wrapped around the lumen in a circular fashion. A third layer of longitudinal muscle (ureters) or obliquely arranged muscle (stomach) is present in some organs. This action and arrangement of smooth muscle layers, causes mixing and/or unidirectional propulsion of materials through the lumen. Movement of substances through lumens by peristalsis occurs in some organs (uterus, urinary bladder, esophagus, stomach, small and large intestines) and ducts (ureters, uterine tubes, vas deferens, bile ducts).
The coordinated manner of contraction that leads to peristalsis, and other forms of coordinated smooth muscle contraction, is due to gap junctions being present between cells of such types of smooth muscle, allowing depolarization to spread from one cell to another. These types of smooth muscles are called single-unit smooth muscle, because all of the cells function as part of a single unit. Smooth muscles that are controlled individually, such as those in the eye, are referred to as multi-unit smooth muscle.
In summary, smooth muscle is found throughout the body around various organs and tracts. Smooth muscle cells have a single nucleus, and are spindle-shaped. Smooth muscle cells are nonstriated, but their sarcoplasm is filled with actin and myosin, along with dense bodies in the sarcolemma to anchor both thin filaments as well as a network of intermediate filaments, which during contraction, are together involved in pulling the sarcolemma toward the fibre’s middle, shortening it in the process.
Practice Questions
Part 1: The two required precedents of skeletal muscular contraction
Part 2: Skeletal muscle fibre contraction and relaxation
Part 3: Cardiac muscle tissue
Part 4: Smooth muscle tissue
Synapse between the axon terminal of a motor neuron and the section of the membrane of a muscle fiber with receptors for the acetylcholine released by the terminal.
Distribution of charge across the cell membrane, based on the charges of ions.
Change in voltage of a cell membrane in response to a stimulus that results in transmission of an electrical signal; unique to neurons and muscle fibres.
Functional division of the nervous system that is concerned with conscious perception, voluntary movement, and skeletal muscle reflexes.
Single process of the neuron that carries an electrical signal (action potential) away from the cell body toward a target cell.
Clear, semi-fluid medium of the cytoplasm, made up mostly of water.
Membrane-bound structure that contains materials within or outside of the cell.
An important neurotransmitter.
Export of a substance out of a cell by formation of a membrane-bound vesicle.
Small gap between cells in a chemical synapse where neurotransmitter diffuses from the presynaptic element to the postsynaptic element.
Change in a cell membrane potential from rest toward zero.
Ion channel that opens because of a change in the charge distributed across the membrane where it is located.
Protein that makes up most of the thin myofilaments in a sarcomere muscle fibre.
Protein that makes up most of the thick cylindrical myofilament within a sarcomere muscle fibre.
Nucleotide containing ribose and an adenine base that is essential in energy transfer.
(In physiology) though under nervous control (usually from the brain), control is not conscious.
Functional division of the nervous system that is responsible for homeostatic reflexes that coordinate control of cardiac and smooth muscle, as well as glandular tissue.
Vasoactive (active on blood vessels) mediator in granules of mast cells and is the primary cause of allergies and anaphylactic shock.